- 1 Overview of canola growth stages
- 2 Canola growth stage 0: Germination to emergence
- 3 Canola growth stage 1: Leaf development
- 4 Canola growth stage 2
- 5 Canola growth stage 3: Stem elongation
- 6 Canola growth stage 4
- 7 Canola growth stage 5: Inflorescence emergence
- 8 Canola growth stage 6: Flowering (including blooming stages)
- 9 Canola growth stage 7: Development of seed
- 10 Canola growth stage 8: Ripening
- 11 Canola growth stage 9: Senescence
- 12 Summary of canola growth stages
- 13 Time to maturity
- 14 Measuring growth and development
- 15 Additional references
- 16 Footnotes
The efficacy of many crop management decisions (such as fertilizer or crop protection productsPesticides (herbicides, insecticides or fungicides) used to protect against or reduce the amount of damage caused by weeds, pest insects or plant diseases. More applications to swath or harvest timing) are dependent on crop growth stage timing. In addition, the impact that climatic factors (such as soil moisture, rainfall, air and soil temperature) have on crops vary depending on the growth stage that they occur at. Therefore it is important to properly identify and understand the canola growth stages to optimize management of this crop.
Overview of canola growth stages
Canola plants grow nearly every day of the growing season, from when they are planted until harvested. Growth begins with the seed, then leaves, stems, flowers, pods and seeds, in a cycle. The length of each phase or stage of growth is influenced by cultivar, fertility and nutrition, moisture, soil and air temperature, day length (photoperiod) and sunlight intensity. Growth and development of a canola plant is continuous but can be divided into easily recognizable growth stages. Air temperature is one of the most important environmental factors regulating growth and development of canola.
A standardized growth stage scale developed by industry research scientists, called the BBCH decimal system, provides an accurate and simplified approach to describing canola growth stages. The BBCH decimal system is comprised of different growth stages (GS) which are each further sub-divided into specific developmental increments 1.
The study of plant growth stage timing as influenced by the genetics of a species or cultivar, the environmental conditions in which the crop is growing in, and the agronomic management of the crop (GxExM) is referred to as phenology. Countless factors and interactions can influence the timing of growth stages, which makes phenology is complex 2.
Canola will move faster or slower through plant growth, cell division and the accumulation of biomass, or plant development, cell specialization and differentiation or the onset and development of reproductive organs, depending on the current environmental conditions the plant is growing in. This includes air and soil temperature, soil moisture that is available to the plant, photoperiod, solar radiation, fertility, and the presence or absence of crop pests. Furthermore, depending on what stage the plant is in determines how sensitive it is to the current environmental conditions. For example, canola that is flowering is much more sensitive to extreme temperatures, like those exceeding 30 degrees Celsius, than it is during vegetative growth.
Canola growth stage 0: Germination to emergence
Oil and protein in the seed provide the energy required for germination, but the seedbed must have sufficient available water, oxygen and adequate temperature for germination to occur.
Water absorption is the first step in germination. Water is the medium and reactant for many biochemical processes. For canola, there is an initial period of rapid water uptake, followed by a lag period, then rapid absorption associated with embryo growth. Since water comes from the soil, the seed must be in close contact with moist soil particles to absorb water. Water absorption by seed cells is influenced by the concentration of inorganic salts and/or organic substances in the soil solution. If the salt concentration is too high, the seed cannot absorb sufficient water for normal germination. This partially explains why a seed may fail to germinate in the fertilizer band or in severely saline soils. Water absorption is also critical for the efficiency of some seed treatments.
Oxygen must also be present for cell respiration to provide adequate energy for germination. Normally, oxygen is a limiting factor only under conditions leading to lower oxygen diffusion rates, such as waterlogged or compacted soils. Soil temperature must be five degrees Celsius for successful germination, and the rate of germination increases from this to about 30 degrees Celsius 3. While water absorption by the seed is not sensitive to temperature, new growth is temperature-dependent because of the effect of temperature on biochemical processes.
Germination is a step-by-step process that includes:
- absorption of water
- activation and synthesis of enzymes
- breakdown of stored food
- transport of breakdown products within the embryo
- initiation of embryo growth
The root grows downward and develops root hairs that anchor the developing seedling. The new stem, or hypocotyl, begins growing up through the soil, pushing two heart shaped leaf-like organs called cotyledons or seed leaves. The seed coat is usually shed in the process. Canola seeds have two cotyledons, and are described as dicotyledonous (or dicots). When exposed to light, the cotyledons unfold and become green.
Other factors that influence germination are seed viability, seed size, soil microorganisms, seed soundness and seed diseases. Viability describes whether the embryo is alive and able to germinate. Soil microorganisms can decay seeds, especially under poor germinating conditions. Seed treatments may help protect the seed and seedling against soil-borne disease infection.
Canola growth stage 1: Leaf development
Roots
Upon emergence, four to 15 days after seeding, the seedling develops a short 1.25 to 2.50 centimetres (0.5 to 1.0 inch) stem. The cotyledons at the top of the hypocotyl (growing point) expand, turn green and provide food to the growing plant. Unlike barley, where the growing point is protected beneath the soil for five to six weeks, the growing point of canola is above the soil, between the two cotyledons. The exposed growing point of canola renders seedlings more susceptible than cereals to spring frost, soil drifting and wind damage, insect feeding and hail. Heat canker may occur when the bare soil temperature becomes so high as to burn the hypocotyl at the soil surface.
Canola plants have a tap root system. Rooting depth varies from three to five centimetres (1.2 to 2.0 inches) at emergence. The root system continues to develop with secondary roots growing outward and downward from the taproot. Root growth is due to cell division and enlargement at the tip of the root. Root growth is relatively constant, averaging nearly two centimetres (0.75 inches) per day, as long as the root has access to adequate soil moisture. Young developing roots quickly become colonized by soil microorganisms – bacteria, fungi, actinomycetes – which provide nutrients, increase plant nutrient uptake, protect against various environmental stressors, and promote positive plant health and growth. They also can help protect the plant from diseases. However, at times, microorganisms that cause disease outcompete beneficial microorganisms, and plant health and productivity declines.
When soil water and nutrients are abundant, the balance of root to stem and leaf growth typically shifts in favour of stem growth at the expense of the roots. When water is limited, the opposite usually occurs. Root and stem growth complement one another by adjusting their relative size to meet the basic requirements of the whole plant in response to climatic and soil conditions.
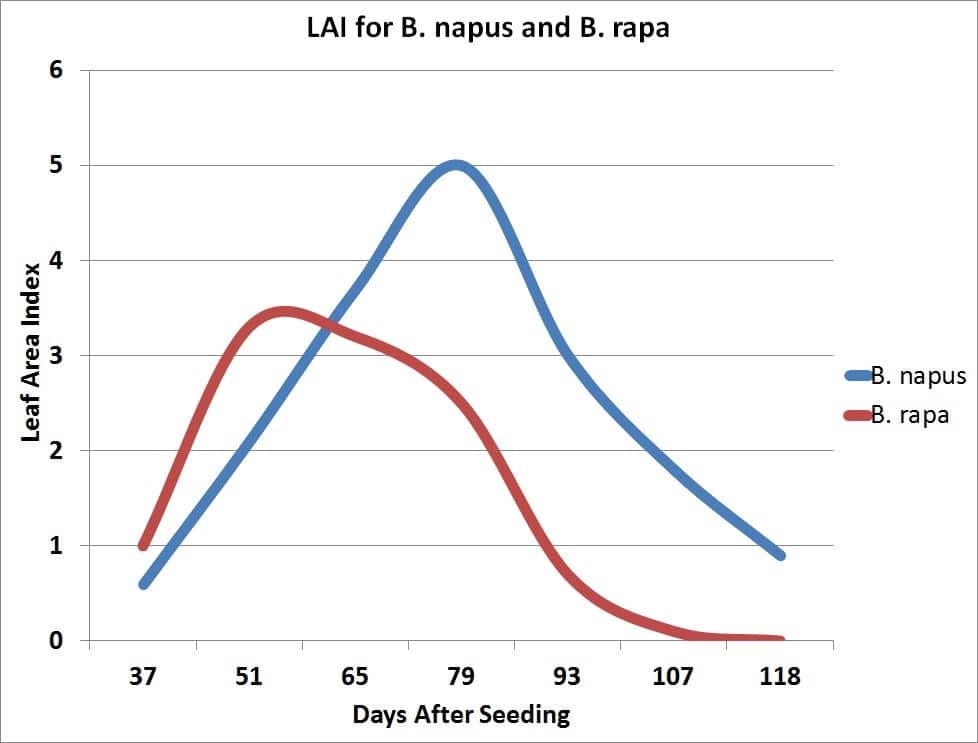
With moisture stressed canola, roots account for about 25 per cent of plant dry matter at stem elongation compared to about 20 per cent for unstressed plants. At peak flowering and maximum stem length, roots will have reached about 85 per cent of their maximum depth. Root depth, like plant height, will vary from 90 to 190 centimetres (36 to 76 inches) and will average about 140 centimetres (56 inches) at maturity. The root system varies with soil type, moisture content, soil temperature, salinity and soil physical structure.
Roots absorb water and nutrients from the soil and transport them upward into the stem. Roots intercept water and nutrients present in the soil pore space that they contact. Factors limiting root penetration through the soil include a high water table, dry soil, soil compaction, weed competition for moisture and nutrients, a salt layer or cool soil temperatures.
As roots grow, they use oxygen and release carbon dioxide. Restricted soil aeration, because of excess water or soil compaction, results in low oxygen, high carbon dioxide, and eventually root death. Moist topsoil with dry sub-soil during the early stage of plant growth promotes a shallow root system. Roots penetrate dry soil only slightly beyond available moisture supplies. Insect and disease species such as root maggot and brown girdling root rot will damage the root and restrict the uptake of water and nutrients.
Leaves
Functionality
Four to eight days after emergence the seedling develops its first true leaves. The first true leaf to develop and fully expand is frilly in appearance. The plant quickly establishes a rosette with older leaves at the base increasing in size and smaller, younger leaves developing in the centre. There is no definite number of leaves produced by a canola plant. A canola plant under optimal growing conditions normally produces nine to 30 leaves on the main stem depending on the cultivar and environmental conditions. The maximum area of individual leaves on the plant in the absence of stress is around 250 square centimetres.
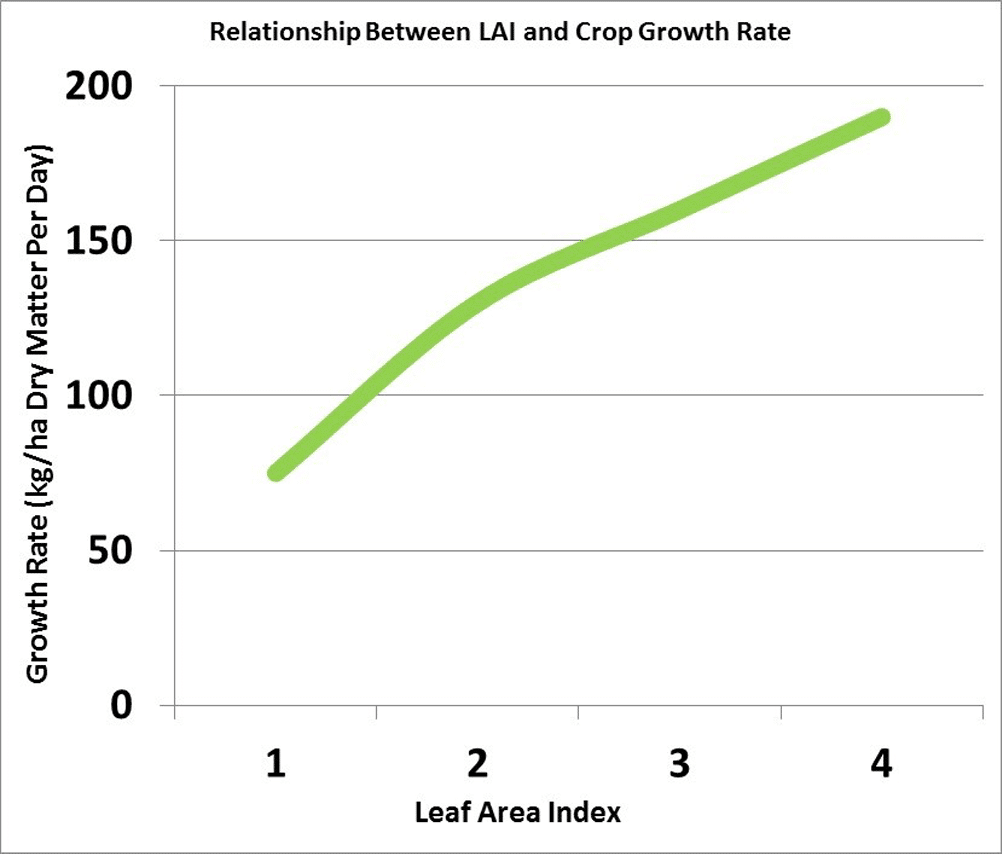
Counting leaves
Count the leaves of a canola plant when it has become visibly separated from the terminal bud. During this rosette growth stage, the stem length remains unchanged although its thickness increases.
Factors affecting leaf development
The growth rate of the crop is closely related to the amount of solar radiation captured by the leaves. Research has shown that canola leaves influence seed yield at early growth stages by influencing the development of the plant’s overall sink capacity, pod set and early seed development. Rapid leaf development also encourages root growth, reduces soil moisture evaporation and competes with unwanted weeds. There is a positive correlation between seed yield and maximum leaf area index (LAI).
Leaf growing rate
Leaf area index (LAI) is a measure of the upper surface area of leaves per unit of ground surface. An LAI of four refers to four square metres of leaf surface area per square metre of ground surface. An LAI of about four is required for the crop canopy to intercept about 90 per cent of the incoming solar radiation. The larger the leaf area the crop can expose to the sun, the more dry matter the crop can accumulate per day. The more dry matter, the greater the potential yield.
Plants in low population density crops (ex. 20 plants per square metre) have a higher LAI than plants in high population density crops (126 plants per square metre). Plants compete with each other for light, soil moisture and nutrients. In uneven germinating crops the leaf area of early emerging plants can become large enough to cause weak, spindly growth or stunting and death of later emerging plants.
Canola growth stage 2
This growth stage (GS 20-29) refers to the development of side shoots (tillering) and occurs in many plant species but it is not applicable to the spring canola cultivarsCultivars are variants in a species developed through the intervention of humans (despite the term 'variety' often being incorrectly used to describe this). Cultivars can be open-pollinated type, hybrid, synthetic, composite, etc. More grown in Canada.
Canola growth stage 3: Stem elongation
Reproductive development begins before flowers are visible to the human eye and can be observed at the microscopic level just prior to bolting. Stems orient leaves to sunlight and air, which are also important photosynthetic structures throughout reproductive development.
Stem elongation (GS 30) overlaps leaf development and normally occurs before the vegetative rosette has stopped growing (GS 19). At or just prior to stem elongation, flower and branch initiation begins. Maximum stem length (GS 39) overlaps flower development and is reached at peak flowering (GS 65). As stems elongate, roots continue to grow deeper. The amount of time spent in the vegetative stage, or days from seeding to first flower, can range from 40 to 60 days, depending on the date of seeding, cultivar, and growing conditions.
Canola plants vary in height, but average between 75 to 175 centimetres (30 to 70 inches). Stem diameter and height are influenced by seeding date, moisture, cultivar, soil fertility status and plant population. Plants in low-density stands will have thicker stems and are more tolerant to lodging. Plants in high-density stands are thinner and more prone to lodging. A lodged stand leads to uneven pod maturity, creates an optimal microenvironment for disease development (such as sclerotinia and alternaria), and can be challenging to swath or harvest.
Canola growth stage 4
This growth stage (GS 40-49) is not important for canola management but applies in the development of harvestable vegetative plant parts such as broccoli or cauliflower.
Canola growth stage 5: Inflorescence emergence
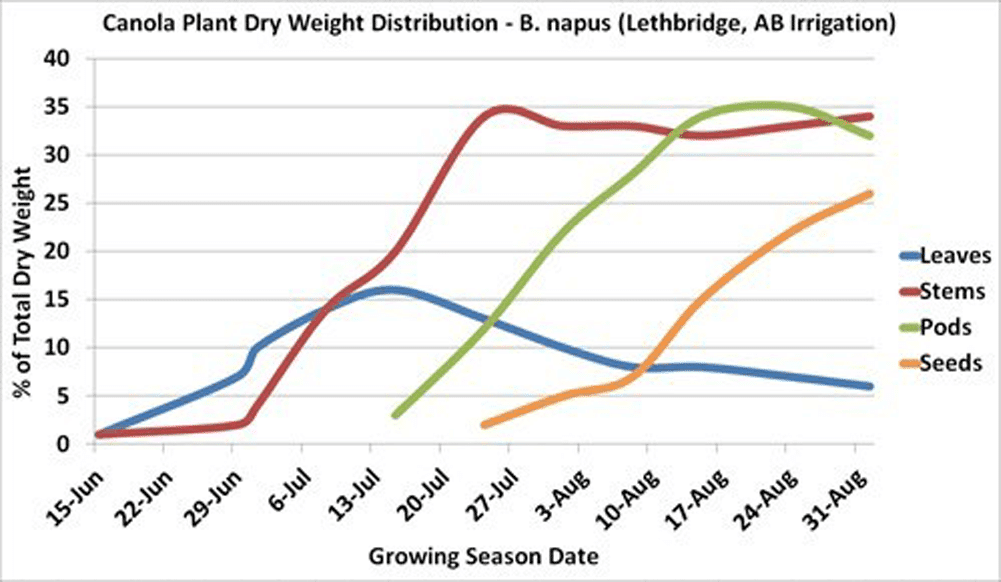
Lengthening days and rising temperatures trigger flower bud formation. Flower development growth stages (GS 50-65) overlap with stem development (GS 30-39). Initially, flower buds (GS 50) remain enclosed during early stem elongation (GS 31) and can only be seen by peeling back young leaves. As the stem elongates a cluster of flower buds can be easily seen from above, but are still not free from leaves. This is known as the green bud stage.
As the stem bolts or elongates, the buds become free from the leaves and the lowest flower stalks extend so that the buds position into a flattened shape. The remaining leaves attached to the main stem unfold as the stem elongates and the small stalks holding the first unopened flower buds become more widely spaced. The lower flower buds are the first to become yellow, signaling the yellow-bud stage.
Secondary branches arise from buds that develop in axils of upper leaves and occasionally from axils of some lower leaves on the main stem. These secondary branches develop one to four leaves and a flower bud cluster. The canola plant initiates many more inflorescences (branches with flower clusters) than it can support, then aborts back according to the plant’s set carrying capacity and environmental conditions. The ability to produce secondary branches is useful as it allows the crop to compensate for less than optimal stand establishment and damage due to hail and pests. Development of branches is not fixed until the end of flowering. Removal of branches by hail can initiate replacement. Environmental stress can reduce the degree of branching, and if the second to fourth primary branches (from the top) are affected, total flower production and therefore total seed yield can be reduced.
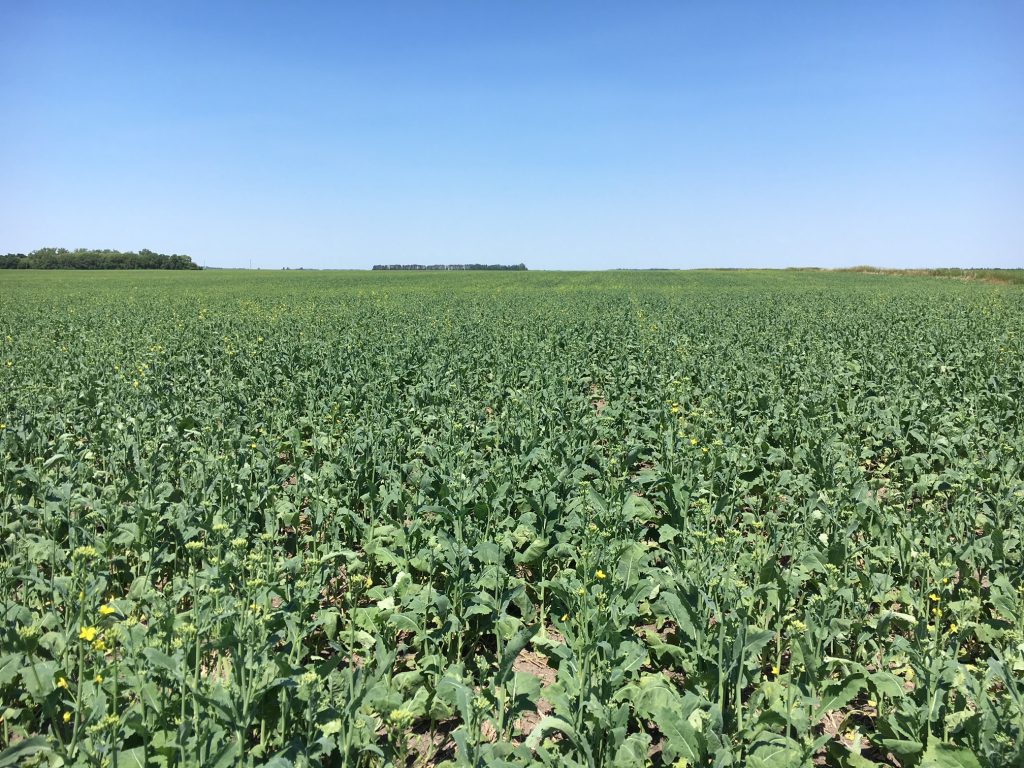
The main stem reaches 30 to 60 per cent of its maximum length just prior to flowering. Also, 30 to 60 per cent of the plant’s total dry matter production will have occurred at this time, depending on environmental conditions.
Maximum leaf area is usually reached near the beginning of flowering and then declines with the loss of lower leaves. The leaves, especially the upper ones at this stage, are the major source of food for the growth of stems and buds. Rapid development and growth of a large leaf area, which is maintained well beyond the start of flowering, strongly influences pod set and early seed development on the main stem and the first few secondary branches. Yield has been found to decrease with leaf area (leaves were removed) at the flowering stage.
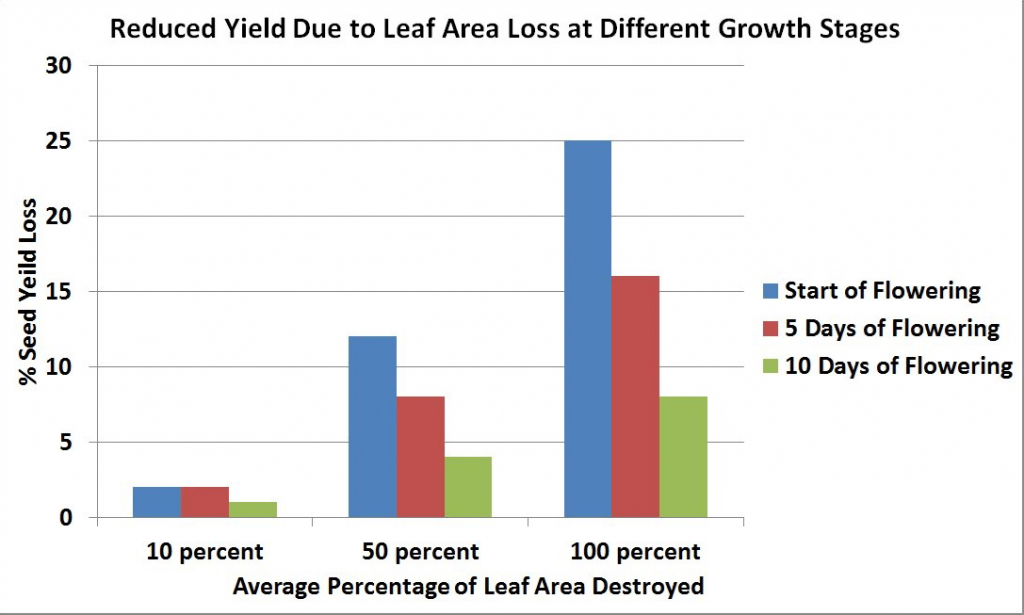
At the start of flowering, leaves are the major source of food for plant growth and their removal results in a large seed yield loss. However, as flowering progresses, leaf area declines and becomes less important as a source of food for plant growth, and its removal at this time results in less seed yield loss than when leaves are removed at earlier stages.
The development and maintenance of a large leaf area after the start of flowering is largely dependent on proper seedbed preparation combined with adequate available soil moisture, air temperature and available nutrients that promote rapid and uniform emergence and growth.
Canola growth stage 6: Flowering (including blooming stages)
Canola cultivarsCultivars are variants in a species developed through the intervention of humans (despite the term 'variety' often being incorrectly used to describe this). Cultivars can be open-pollinated type, hybrid, synthetic, composite, etc. More are self-pollinated and do not need pollinating agents such as wind and insects. About 70 to 80 per cent of the seed produced is from self-pollination. The crop is very attractive to bees (with the yellow flowers), but their presence does not always increase yield. However, some research has reported that bees can cause seed set to occur earlier, resulting in shorter, more compact plants that ripen more uniformly.
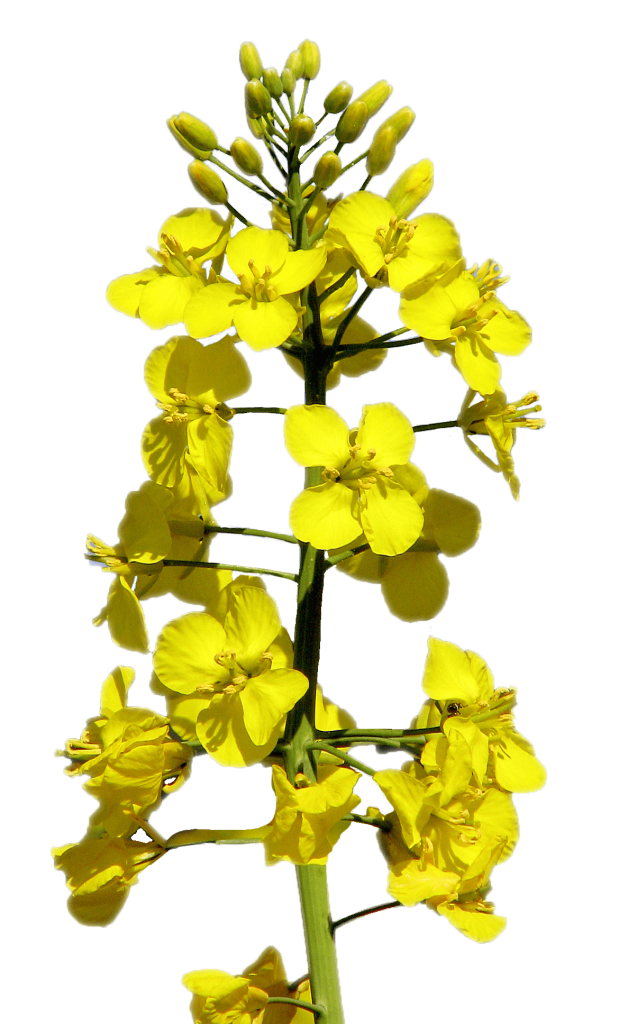
Photo credit Scott Gillies
Flowering duration
Flowering begins with the opening of the lowest bud on the main stem and continues upward with three to five or more flowers opening per day. Flowering at the base of the first secondary branch begins two to three days after the first flower opens on the main stem.
Under reasonable growing conditions, flowering of the main stem will continue from 14 to 21 days for both species. Full plant height (GS 39) is reached at peak flowering (GS 65) due to the overlap of growth stages.
Pollination and fertilization
Flowers begin opening early in the morning and, as the petals completely unfold, pollen is shed and dispersed by both wind and insects. Flowers remain receptive to pollen for up to three days after opening. If favourable warm, dry weather occurs, nearly all the pollen is shed the first day the flower opens. In the evening, the flower partially closes and opens again the following morning. Fertilization occurs within 24 hours of pollination. After pollination and fertilization, the flower remains partially closed and the petals wilt and drop (two to three days after the flower opened). The young pod becomes visible in the centre of the flower a day after petals drop.
Development during flowering
During flowering, the branches continue to grow longer as buds open into flowers and as flowers develop into pods. In this way, the first buds to open become the pods lowest on the main stem or secondary branches. Above them are the open flowers, and above them, the buds which are yet to open. All buds that develop into open flowers on the main stem will likely be visible within three days after the start of flowering.
Canola plants initiate more buds than they can develop into productive pods. The flowers open, but the young pods fail to enlarge and elongate, and eventually fall from the plant.
The abortion of some flowers and pods is natural. Both flowers and seeds can undergo substantial abortion depending on the carrying capacity established by leaf, stem, and branch growth, plus environmental stress imposed during flowering and seed set. During flowering, the plant can adjust yield based on the number of flowers produced and pollinated. Under stress, the number of branches that produce flowers may be reduced and the number of flowers on each branch may decline. Flowers that are open during heat stress may fail to pollinate. Normally, fertility of flowers that open later will be unaffected if stress has been alleviated. Areas on the main stem or branches with no pod development are symptoms of stress. Under severe stress, loss of unopened buds increases, signaling the end of flowering. Research from Agriculture and Agri-Food CanadaAgriculture and Agri-Food Canada is a department of the Government of Canada. More (AAFCAgriculture and Agri-Food Canada is a department of the Government of Canada. More) reported that ambient temperature above 29.5 degrees Celsius from bolting to the end of flower imposes heat stress and can reduce seed yield 6.
Moisture stress, drought or in excess, can also impact seed yield during this time. Lower yields have been reported in studies where moisture was limited during flowering, compared to the stem elongation and seed fill stages 7. If severe stress occurs at early flowering, the plant may resume flowering through increased branching if favourable conditions return.
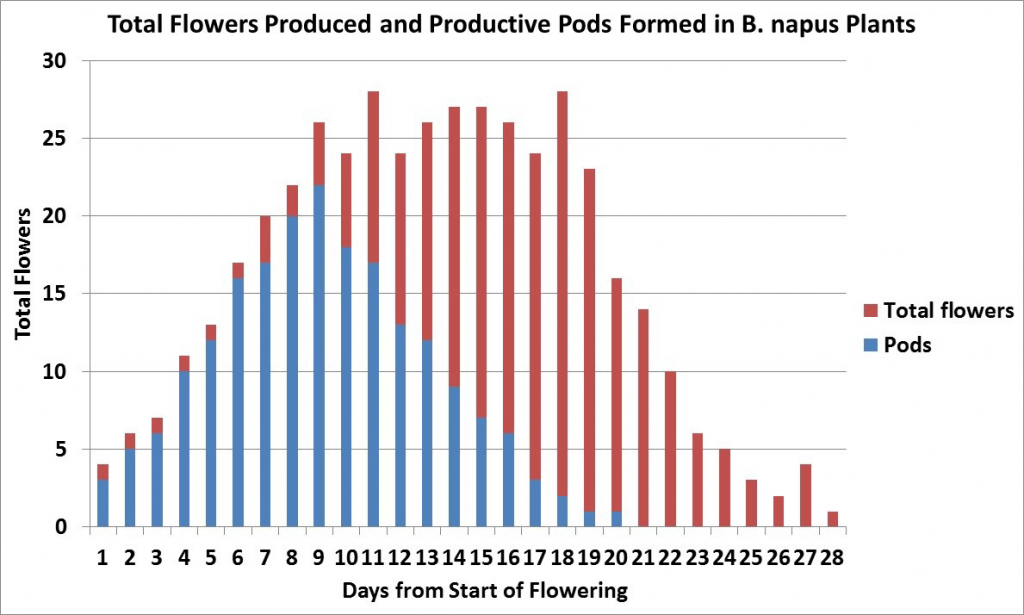
Another research project from AAFCAgriculture and Agri-Food Canada is a department of the Government of Canada. More has shown that only 40 to 55 per cent of the flowers produced on a plant develop productive pods, which are retained until harvest 8. In this study, which was conducted in a dry year, most productive pods were from flowers that opened within the first 15 days of flowering on the main stem and first three secondary branches. Later flowers and pods on all branches aborted. Under more favourable growing conditions more flowers and pods would have been produced but the percentage of abortions would have been similar.
Additional flower functionality
At the peak of flowering canola produces a bright yellow layer of flowers, at least 30 centimetres (12 inches) thick, which forms an effective reflecting and absorbing surface for solar radiation at the top of the crop. Canola flowers reflect or absorb more incoming radiation than vegetative canopies, or the photosynthetic active tissues of the plant 6. Furthermore, apetalous canola cultivarsCultivars are variants in a species developed through the intervention of humans (despite the term 'variety' often being incorrectly used to describe this). Cultivars can be open-pollinated type, hybrid, synthetic, composite, etc. More (cultivarsCultivars are variants in a species developed through the intervention of humans (despite the term 'variety' often being incorrectly used to describe this). Cultivars can be open-pollinated type, hybrid, synthetic, composite, etc. More that have smaller, fewer, or no flowers) have greater solar radiation interception during the normal flowering period and therefore greater photosynthetic capacity than cultivarsCultivars are variants in a species developed through the intervention of humans (despite the term 'variety' often being incorrectly used to describe this). Cultivars can be open-pollinated type, hybrid, synthetic, composite, etc. More with flowers, because petals will block incoming radiation to the canopy 9.
The main reason for the decrease in leaf area index (LAI) after floral initiation is the reduction of radiation into the leaf canopy caused by flower petals. This shading results in senescence of active green leaves. Therefore, apetalous cultivarsCultivars are variants in a species developed through the intervention of humans (despite the term 'variety' often being incorrectly used to describe this). Cultivars can be open-pollinated type, hybrid, synthetic, composite, etc. More should have a greater photosynthetic capacity through increased solar radiation to the crop canopy at the critical stage for developing pods and seeds.
Canola growth stage 7: Development of seed
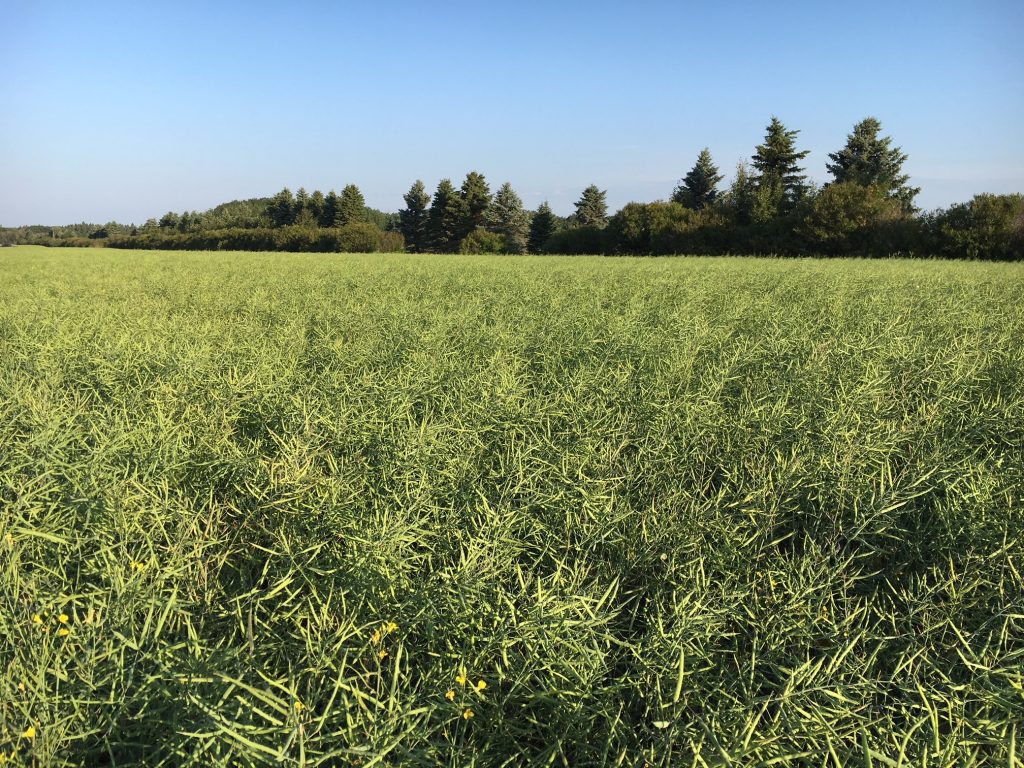
By mid-flower, when lower pods have started elongating, the stem becomes the major source of food for plant growth, with a reduced amount from the declining leaves and a small amount from the developing pods. There is competition for the food supply between flowers and pods on the same branch, as well as between branches. The early developed pods have a competitive advantage over later formed pods. Flowering on the later developing secondary branches may continue for some time after the main stem has finished flowering. Older pods at the base of the flowering branches are well along in development while new flowers are still being initiated at the tips. At this stage, the stem and pod walls are both major sources of food for seed growth since the pod photosynthetic surface area has greatly increased.
During the first couple of weeks of seed development, the seed coat expands until the seed is almost full size. The seed at this stage is somewhat translucent and resembles a water-filled balloon. The seed’s embryo now begins development and grows rapidly within the seed coat to fill the space previously occupied by fluid; seed weight increases.
Any stress leading to a change in the supply of nutrients can abort pods or reduce the number of seeds in each pod. The stress may be internal where the plant is unable to take up soil water available to it or to generate food supplies necessary for seed filling. The stress can be external where soil water is limited or temperatures excessive for optimal crop development.
The number of seeds that develop in each pod will be influenced by the availability of plant food supplies at the time when seed expansion occurs. Lack of plant food supplies at this growth stage will result in smaller pods with fewer, lighter seeds, especially in the later secondary branches and tops of branches. Substantial stress at seed expansion leads to shorter pods and/or lack of expansion around missing seeds. Segments of the pods will not expand normally with little or no sign of seed remnants inside the pod.
Plants under stress redirect food supplies from stems and pods to those seeds that are left. The only way a plant can respond to more favourable conditions late in the growing season is by producing larger seeds. When severe stress occurs later in the seed filling process, the pod appears normal because the seed expanded normally and then started to die off, resulting in a shriveled seed coat with little or no evidence of having started the seed filling process.
Once seed expansion is complete, seeds are more resistant to loss from stress, but losses can occur if stress is severe. The plant attempts to redirect food supplies to seeds that continue filling. Pods show no external signs of stress, but affected seeds may be visibly shriveled within the pod. Even where shriveling is not evident, due to reduced food supplies, seed size will be smaller and a larger portion of seeds will have wrinkled seed coats. This is affected by temperature and moisture. Canola is sensitive to drought at this stage.
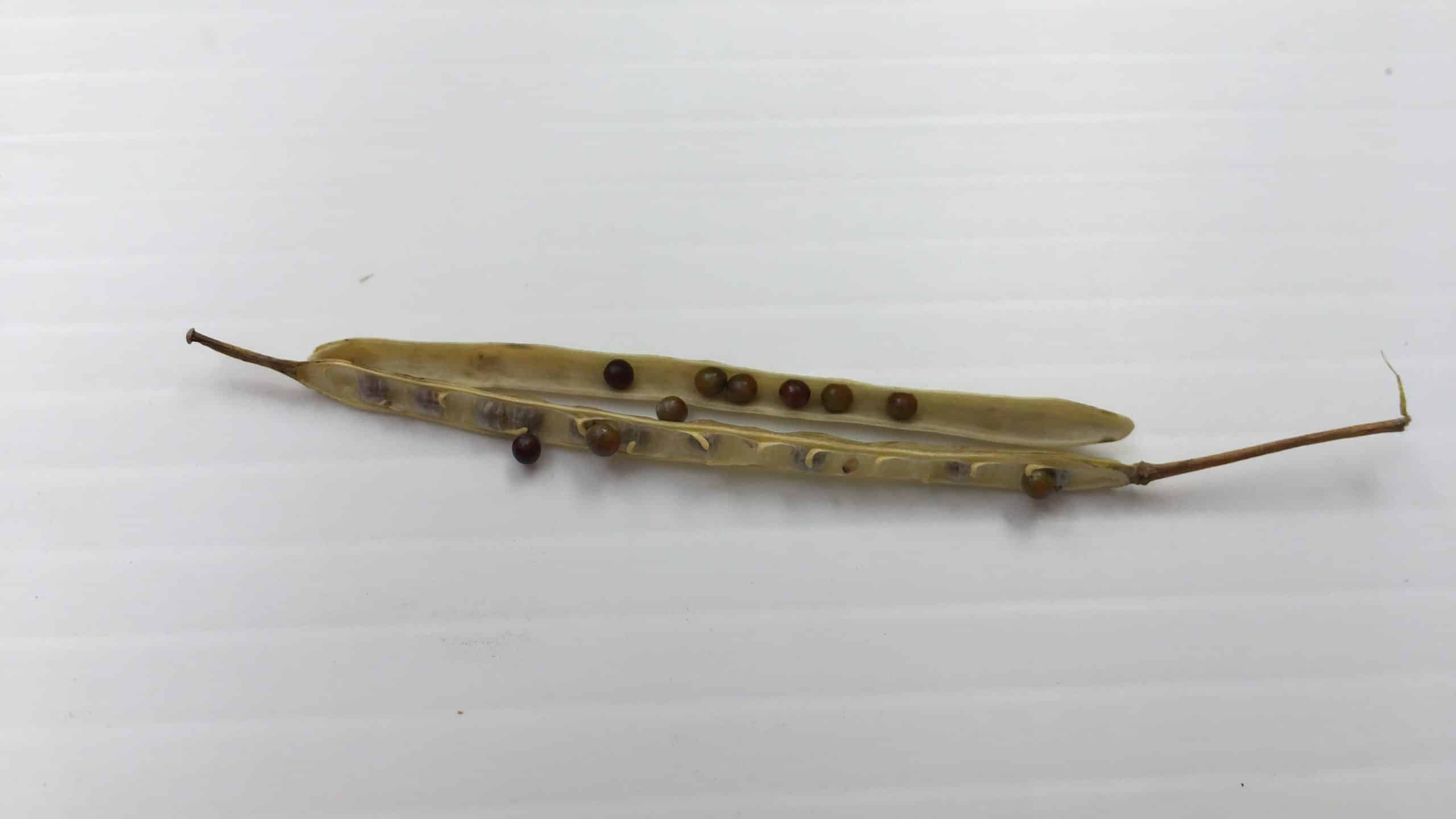
The pod is divided internally into two halves by a membrane, which runs the full length of the pod. Normally a pod contains 15 to 40 seeds. Canola seeds are typically 3.5 to 5.5 grams per 1,000 seeds (182,000 to 286,000 seeds per kilogram or 83,000 to 130,000 seeds per pound).
Canola growth stage 8: Ripening
At the stage where seeds in the lower pods have turned green, most of the leaves on the plant have yellowed and fallen from the plant. The pod walls have become the major source of food although the stem is still an important source. The pods, besides being major food producers, are also major food users from other sources for seed development.
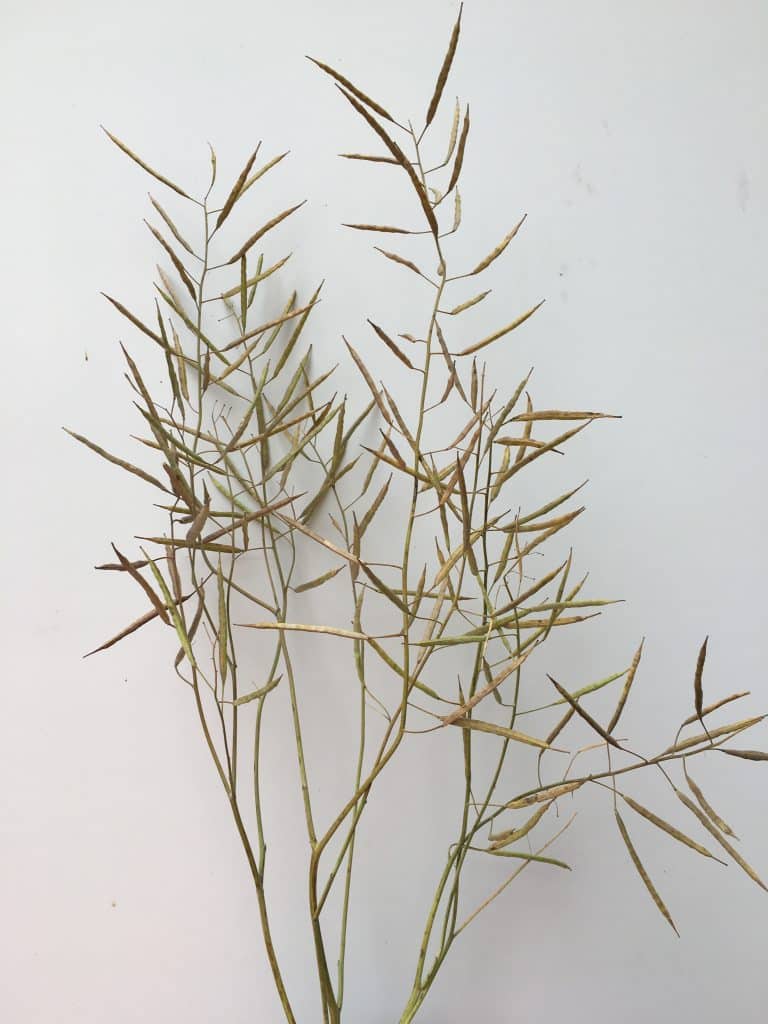
About 35 to 45 days after the first flower opens, seed filling is complete. The firm green seed has adequate oil and protein reserves to support future germination and seedling growth and has now reached physiological maturity. The stems and pods turn yellow and progressively become brittle as they dry.
Usually, the earliest formed pods are the largest and develop more and larger seeds. Immature seeds, when filled, contain about 40 to 45 per cent moisture content. The seed coat then begins to turn from green to yellow or brown, depending on the cultivar. Seed moisture is rapidly lost at a rate of two to three per cent or more per day, depending on growing conditions. At 40 to 60 days after first flower, or 25 to 45 days after the end of flowering, the seeds in the lower pods will have ripened and fully changed colour. As the seed coat changes colour so does the seed. The embryo, which fills the entire seed, begins to lose its green colour. When completely mature the seed is uniformly bright yellow in colour. When 30 to 40 per cent of the seeds on the main stem of a plant have begun to change seed coat colour (black or yellow), seeds in the last formed pods are in the last stages of filling. The majority of seeds have reached physiological maturity when average seed moisture content is from about 30 to 35 per cent. This is the optimum stage for swathing. Swathing before physiological maturity can result in reduced seed yield and quality due to incomplete seed development.
Although the potential number of pods per plant and seeds per pod are set at flowering, the final number is not established until a later stage. Seed filling requires adequate soil moisture and nutrients. Seed abortion, or reduction in seed weight, can be caused by anything that interferes with plant functions during this time.
In canola, the seed accounts for about 23 to 31 per cent of the total plant dry matter produced, depending upon growing conditions. The leaves, stems, and especially pod surface areas must be kept free from disease, insect and weather damage. Anything that stresses or reduces the food production capacity of these plant surfaces may lead to a reduction in seed yield.
Canola growth stage 9: Senescence
When seed in all pods have changed colour, the plant dies. At this point there is potential for mature pods to shatter (split open along the centre membrane) and lose the seed.
Summary of canola growth stages
The life cycle of the canola plant is divided into seven principle stages. Learning the growth stages will improve the ability to make the critical management decisions at each development stage, especially those that can only be done or are optimal at certain stages. Each growth stage covers a portion of the development of the plant. However, the beginning of each stage is not dependent on the completion of the preceding stage. Several growth stages tend to overlap. From the onset of budding each growth stage is determined by examining the main flowering (terminal) stem. The timing and occurrence of the different growth stages will vary with environmental conditions, crop inputs and cultivar. When two stages overlap, use the growth stage number for the stage that is represented by greater than 50 per cent of the crop.
Time to maturity
Time to maturity, or days from seeding to harvest, is a measurement of the total duration of canola growth. Time to maturity varies considerably, and depends on environment, crop inputs, cultivar and date of seeding.
Since the mid-1990s, many new canola cultivarsCultivars are variants in a species developed through the intervention of humans (despite the term 'variety' often being incorrectly used to describe this). Cultivars can be open-pollinated type, hybrid, synthetic, composite, etc. More have been made commercially available to farmers. Some of these are adapted to specific growing environments. CultivarsCultivars are variants in a species developed through the intervention of humans (despite the term 'variety' often being incorrectly used to describe this). Cultivars can be open-pollinated type, hybrid, synthetic, composite, etc. More require fewer days to mature when grown in southern canola growing areas because of the greater heat units available, or warmer temperatures than in northern canola production areas. In the far north, the longer day lengths tends to offset lower heat units available.
The Canola Performance Trials provide data on days to maturity (along with other results) for all the cultivarsCultivars are variants in a species developed through the intervention of humans (despite the term 'variety' often being incorrectly used to describe this). Cultivars can be open-pollinated type, hybrid, synthetic, composite, etc. More tested, in several growing environments (across western Canada). It is important to understand that cultivarsCultivars are variants in a species developed through the intervention of humans (despite the term 'variety' often being incorrectly used to describe this). Cultivars can be open-pollinated type, hybrid, synthetic, composite, etc. More grow and develop differently, or at different rates, among growing environments, so keep this in mind when consulting trial data.
Measuring growth and development
Measuring crop growth and development through the growing season can be beneficial for farmers, agronomists, and researchers and can be used to explain seed yield differences among management practices, cultivarsCultivars are variants in a species developed through the intervention of humans (despite the term 'variety' often being incorrectly used to describe this). Cultivars can be open-pollinated type, hybrid, synthetic, composite, etc. More, environments, and rates of crop inputs.
Crop growth, the rate in which the crop is accumulating biomass, can be measured using different methods. It is almost always best to record these measurements continuously through the growing season, so a timeline of measurements can be analyzed at the end of the crop’s life cycle. Measurements like plant height, LAI, and plant or root biomass are common measurements used to analyze plant growth.
Measuring the rate of development through the growing season involves recording the calendar date of when growth stages occurred. Record a field as a growth stage when at least 50 per cent of the field has reached that stage. Once a calendar date timeline of growth stages has been recorded, calculate the amount of heat units, like the growing degree day (GDD), between stages or over the entire life cycle of the crop. Using ‘days’ as a unit of measurement for rate of development can be inaccurate because each ‘day’ has different environmental conditions than the next. Furthermore, it can be helpful to examine environmental conditions during specific growth stage intervals when explaining yield results at the end of the growing season. This is done most effectively when the dates of growth stages have been recorded, and this timeline of growth stages are paired with local weather data.
Growing Degree Days
The Growing Degree Day or GDD is an equation used to measure and quantify temperature over time. The GDD is calculated daily and is often summed over many days. A GDD for a given day cannot be negative, as this implies negative growth, and should be set to 0 if it is calculated to be a negative value. The GDD is the average between the daily maximum temperature (Tmax) and daily minimum temperature (Tmin) and then it is subtracted by a base temperature (Tbase), which is specific to the crop or species it is used for. A base temperature of 5 degrees Celsius should be used for canola in Canada 10.
GDD = [(Tmax + Tmin)/2] – Tbase
Footnotes
- Uwe Meier. 2001. BBCH Monograph: Growth stages of mono-and dicotyledonous plants. 2 Edition. Federal Biological Research Centre for Agriculture and Forestry, 28-32.[↩]
- Ort, N. 2022. How environment influences canola growth stage timing. Canola Digest – January 2022 edition.[↩]
- Derakhshan, A., Bakhshandeh, A., Siadat, S.A. allah, Moradi-Telavat, M.R., & Andarzian, S.B. 2018. Quantifying the germination response of spring canola (Brassica napusAlso referred to as Argentine canola, it is the species of canola currently commonly grown in Canada. More L.) to temperature. Ind. Crops Prod., 122, 195–201. Elsevier. doi:10.1016/j.indcrop.2018.05.075[↩]
- Clarke, J.M., and Simpson, G.M. 1978. Growth Analysis of Brassica napusAlso referred to as Argentine canola, it is the species of canola currently commonly grown in Canada. More CV. Tower. Can. J. Plant Sci., 58, 587–595.[↩][↩]
- Major, D.J. 1977. Analysis of Growth of Irrigated Rape. Can. J. Plant Sci., 57, 193–197. doi:10.4141/cjps77-027[↩]
- Morrison, M.J., and Stewart, D.W. 2002. Heat Stress during Flowering in Summer Brassica. Crop Sci., 42, 797–803.[↩][↩]
- Birunara, A., Shekari, F., Hassanpouraghdam, M.B., Khorshidi, M.B., & Esfandyari, E. 2011. Effects of water deficit stress on yield, yield components and phenology of canola (Brassica napusAlso referred to as Argentine canola, it is the species of canola currently commonly grown in Canada. More L.) at different growth stages. J. Food, Agric. Environ., 9, 506–509.[↩]
- McGregor, D.I. 1981. Pattern of Flower and Pod Development in Rapeseed. Can. J. Plant Sci., 61, 275–282. doi:10.4141/cjps81-040[↩]
- Jiang, L. 2007. Breeding for Apetalous Rape: Inheritance and Yield Physiology. Adv. Bot. Res., 45, 217–231. doi:10.1016/S0065-2296(07)45008-X[↩]
- Morrison, M.J., McVetty, P.B.E., & Shaykewich, C.F. 1989. The Determination and Verification of a Baseline Temperature for the Growth of Westar Summer Rape. Can. J. Plant Sci., 69, 455–464[↩]
- Allen, E.J. and Morgan, D.G. 1975. A quantitative comparison of the growth, development and yield of different varieties of oilseed rape. J. Agric. Sci. (Camb.), 85, 159-174.[↩]
- Allen, E.J., Morgan, D.G. & Ridgman, W.J. 1971. A physiological analysis of the growth of oilseed rape. J. Agric. Sci. (Camb.), 77, 339-341.[↩]
- Bouttier, C. and Morgan, D.G. 1992. Development of oilseed rape buds, flowers and pods in vitro. Journal of Experimental Botany, 43, 1089-1096.[↩]
- Campbell, D.C. and Kondra, Z.P. 1977. Growth pattern analysis of three rapeseed cultivarsCultivars are variants in a species developed through the intervention of humans (despite the term 'variety' often being incorrectly used to describe this). Cultivars can be open-pollinated type, hybrid, synthetic, composite, etc. More. Can. J. Plant Sci., 57, 707-712.[↩]
- Campbell, D.C. and Kondra, Z.P. 1978. Relationships among growth patterns, yield components and yield of rapeseed. Can. J. Plant. Sci., 58, 87-93.[↩]
- Chongo, G. and McVetty, P.B.E. 2001. Relationship of physiological characters to yield parameters in oilseed rape (Brassica napusAlso referred to as Argentine canola, it is the species of canola currently commonly grown in Canada. More L.). Can. J. Plant Sci., 81, 1-6.[↩]
- Clarke, J.M. 1979. Intra-plant variation in number of seeds per pod and seed weight in Brassica napusAlso referred to as Argentine canola, it is the species of canola currently commonly grown in Canada. More ‘Tower’. Can. J. Plant Sci., 59, 959-962.[↩]
- Clarke, J.M. and Simpson, G.M. 1978. Influence of irrigation and seeding rates on yield and yield components of Brassica napusAlso referred to as Argentine canola, it is the species of canola currently commonly grown in Canada. More cv. Tower. Can. J. Plant Sci., 58, 731-737.[↩]
- Copyright by Academic Press Canada, 1983[↩]
- Daniels, R.W., Scarisbrick, D.H. & Smith, L.J. 1986. Oilseed rape physiology. In: Scarisbrick, D.H. and Daniels, R.W. (eds) Oilseed Rape. Collins, London, 83-126.[↩]
- Eisikowitch, D. 1981. Some aspects of pollination of oil seed rape (Brassica napusAlso referred to as Argentine canola, it is the species of canola currently commonly grown in Canada. More L.). J. Agric. Sci. (Camb.), 96, 321-326.[↩]
- Evans, E.J. 1984. Pre-anthesis growth and its influence on seed yield in winter oilseed rape. Aspects of Applied Biology, 6, 81-90.[↩]
- Freyman, S., Charnetski, W.A. & Crookston, R.K. 1973. Role of leaves in the formation of seed in rape. Can. J. Plant Sci., 53, 693-694.[↩]
- Harper, F.R. and Berkenkamp, B. 1975. Revised growth-stage key for Brassica campestris and B. napusAlso referred to as Argentine canola, it is the species of canola currently commonly grown in Canada. More. Can. J. Plant Sci., 55, 657-658.[↩]
- Kjellstrom, C. 1993. Comparative growth analysis of Brassica napusAlso referred to as Argentine canola, it is the species of canola currently commonly grown in Canada. More and Brassica junceaAlso referred to as brown mustard, it is a minor crop (from the Cruciferae or Brassicaceae plant family, commonly known as the mustard family) grown in Canada. More under Swedish conditions. Can. J. Plant Sci., 71, 795-801.[↩]
- Lancashire, P.D., Bleiholder, H., Van Den Boom, T. Langeluddeke, P., Stauss, S., Weber, E. & Witzenberger, A. 1991. A uniform decimal code for growth stages of crops and weeds. Ann. Appl. Biol., 119, 561-601.[↩]
- Major, D.J., Bole, J.B. & Charnetski, W.A. 1978. Distribution of photosynthates after 14CO2 assimilation by stems, leaves, and pods of rape plants. Can. J. Plant Sci., 587, 783-787.[↩]
- Mendham, N.J., Rao, M.S.S. & Buzza, G.C. 1991. The apetalous flower character as a component of a high yielding ideotype. Proceedings of the eighth International Rapeseed Congress. Saskatoon, 596-600.[↩]
- Mendham, N.J. and Salisbury, P.A. 1995. Physiology – crop development, growth and yield. In: Kimber, D.S. and McGregor, D.I. (eds) Production and Utilization of Brassica Oilseeds. CAB International, Oxford. 11-65.[↩]
- Mendham, N.J., Shipway, P.A. & Scott, R.K. 1981. The effects of seed size, autumn nitrogen and plant population density on the response to delayed sowing in winter oil-seed rape (Brassica napusAlso referred to as Argentine canola, it is the species of canola currently commonly grown in Canada. More). J. Agric. Sci. (Camb.), 96, 417-428.[↩]
- Morrison, M.J., Stewart, D.W. & McVetty, P.B.E. 1992. Maximum area, expansion rate and duration of summer rape leaves. Can. J. Plant Sci., 72, 117-126.[↩]
- Pechan, P.A., and Morgan, D.G. 1985. Defoliation and its effects on pod and seed development in oil seed rape (Brassica napusAlso referred to as Argentine canola, it is the species of canola currently commonly grown in Canada. More L.). Journal of Experimental Botany, 36, 458-468.[↩]
- Rao, M.S.S., Mendham, N.J. & Buzza, G.C. 1991. Effect of the apetalous flower character on radiation distribution in the crop canopy, yield and its component in oilseed rape (Brassica napusAlso referred to as Argentine canola, it is the species of canola currently commonly grown in Canada. More). Journal of Agricultural Science (Cambridge), 117, 189-196.[↩]
- Rood, S.B., Major, D.J. & Charnetski, W.A. 1984. Seasonal changes in 14CO2 assimilation and 14C translocation in oilseed rape. Field Crops Res., 8, 341-348.[↩]
- Smith, L.J. and Scarisbrick, D.H. 1990. Reproductive development in oilseed rape (Brassica napusAlso referred to as Argentine canola, it is the species of canola currently commonly grown in Canada. More cv. Bienvenu) Annuals of Botany, 65, 205-212.[↩]
- Strauss, R. 1994. Compendium of growth stage identification keys for mono- and dicotyledonus plants – Extended BBCH scale. Ciba- Geigy AG.[↩]
- Sylvester-Bradley, R. and Makepeace, R.J. 1984. A code for stages of development in oilseed rape (Brassica napusAlso referred to as Argentine canola, it is the species of canola currently commonly grown in Canada. More L.). Aspects of applied Biology, 6, 399-419.[↩]
- Tayo, T.O. and Morgan, D.G. 1975. Quantitative analysis of the growth, development and distribution of flowers and pods in oil-seed rape (Brassica napusAlso referred to as Argentine canola, it is the species of canola currently commonly grown in Canada. More L.). Journal of Agricultural Science (Cambridge), 85, 103-110.[↩]
- Tayo, T.O. and Morgan, D.G. 1979. Factors affecting flower and pod development in oil-seed rape (Brassica napusAlso referred to as Argentine canola, it is the species of canola currently commonly grown in Canada. More L.). Journal of Agricultural Sciences (Cambridge), 92, 363-373.[↩]
- Thurling, N. 1974a. Morphophysiological determinants of yield in rapeseed (Brassica campestris and Brassica napusAlso referred to as Argentine canola, it is the species of canola currently commonly grown in Canada. More L.). I. Growth and morphological characters. Australian J. Agricultural Research, 25, 697-710.[↩]
- Thurling, N. 1974b. Morphophysiological determinants of yield in rapeseed (Brassica campestris and Brassica napusAlso referred to as Argentine canola, it is the species of canola currently commonly grown in Canada. More L.). II. Yield components. Australian J. Agricultural Research, 25, 711-721.[↩]
- Williams, I.H. 1978. The pollination requirements of swede rape (Brassica napusAlso referred to as Argentine canola, it is the species of canola currently commonly grown in Canada. More L.) and of turnip rape (Brassica campestris L.). Journal of Agricultural Science (Cambridge), 91, 343-348.[↩]
- Williams, I.H., Martin, A.P. & White, R.P. 1987. The effect of insect pollination on plant development and seed production in winter oil-seed rape (Brassica napusAlso referred to as Argentine canola, it is the species of canola currently commonly grown in Canada. More L.). Journal of Agricultural Science (Cambridge), 109, 135-139.[↩]
- Yates, D.J. and Steven, M.D. 1987. Reflexion and absorption of solar radiation by flowering canopies of oil-seed rape (Brassica napusAlso referred to as Argentine canola, it is the species of canola currently commonly grown in Canada. More L.). Journal of Agricultural Science (Cambridge), 109, 495-502.[↩]